|
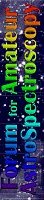
|
Amateur Astronomical Spectroscopy:
Highlights of what is Currently Achievable
by Dale E. Mais
Mais Observatory, Valley Center, CA
|
Introduction
Spectroscopy had its beginnings in the later half of the 19th century where it was
primarily the domain of the amateur working out of his private observatory. As we
entered the 20th century with a greater emphasize on astrophysics, this area of
research shifted toward the professional astronomer working out of world-class
observatories. This shift was primarily driven by the increasing costs and skills
required to do state-of-the-art spectroscopy and the requirement for large telescopes
due to film based detection of a spectrum. Once again we are experiencing a shift
where the amateur can make contributions to the area of spectroscopy. This is due
to both the use of more sensitive CCD detectors and the recent availability of
powerful and versatile spectrometers aimed at the amateur community. I will focus
on the instrument produced by Santa Barbara Instrument Group (SBIG), the Self-Guided
Spectrometer (SGS). In the past, due to the limitations of film based detection and
amateurs were limited to obtaining
spectra of only the brightest stars and nebulae. The SGS allows spectra to be
obtained with only modest aperture instruments of stars down to 10-12th magnitude.
Equipment
My primary instrument for spectroscopy and the evaluation of the SGS is a
Celestron 14, which has had a Byers retrofitted drive system. The spectrometer is
linked to the telescope with a focal reducer giving a final f6 ratio. The CCD camera
attached to the spectrometer is the SBIG ST-7E with 9-µm pixel size. The SGS
instrument appeared on the market during the later half of 1999 and was aimed at
a sub group of amateurs with special interest in the field of spectroscopy [1].
The instrument is shown in Figure 1 with a number of features pointed out and the
path of light indicated.
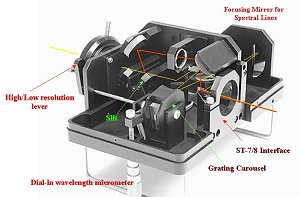
|
Figure 1.
The Santa Barbara Instrument Group Self-Guiding Spectrometer
showing various features of the instrument and the optical path. The yellow
(lighter) path shows the route followed by light passing through the slit to
the grating and ultimately to the imaging chip of the ST-7/8 camera. The red
(heavier line) path is the route followed by the light not passing through
the slit and ending at the guiding chip of the same camera.
(Click on image for enlarged illustration.)
|
The instrument features several very novel features. In
conjunction with SBIG CCD cameras, the SGS is self-guiding in that it keeps the
image of an object locked onto the entrance slit, which allows for long exposures
to be taken. The light from the telescope reaches the entrance slit, which can be 18 or
72-µm wide. The light passes through the slit and reaches the grating and ultimately
the CCD cameras imaging chip. The remaining field of view is observed on the guiding
CCD chip of the camera and allows the viewer to select a field star to guide upon once
the object of interest is centered on the slit. In this book chapter only results
obtained using the 18-µm slit will be presented. The wider slit option allows
the spectra of fainter objects to be obtained at the expense of resolution. This
would be particularly of interest to those interested in measuring the red shifts
of more distant and thus fainter objects since the wider slit permits an additional
2 magnitudes of penetration.
The SGS features a dual grating carousal, which, with the flip of a lever, allows
dispersions both in the low-resolution mode (~4 Angstroms/pixel, ~400 Angstroms/mm)
or higher resolution mode (~1 Angstrom/pixel, ~100 Angstroms/mm). In the
low-resolution mode, about 3200 Angstrom coverage is obtained whereas in the
high-resolution mode, about 800 Angstroms. The particular region of the spectrum
is determined by a micrometer dial and is set by the user. The overall wavelength
range of the unit is from approximately 10000 to 3500 Angstroms. Spectra are
obtained using CCDOPS software and are analyzed using the software package SPECTRA,
both software packages from SBIG, which allows for wavelength calibration.
Wavelength calibration was carried out using emission lines from Hydrogen and/or
Mercury gas discharge tubes. These tubes along with many others are available from
Edmund’s Scientific. The light from these standard lamps is fed to the spectrometer
by means of fiber optic leads into an opal window at the
bottom of the spectrometer. This window has been modified slightly by the author
such that the fiber optic lead remains permanently in position, as does their
position at the source emission tubes. Spectral images obtained were further
processed using MaxIm software package [2].
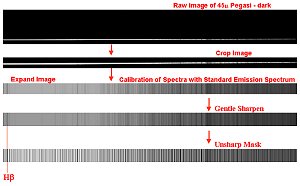
|
Figure 2.
Example of processing steps involved in spectral analysis. The raw spectrum
is only a few pixels wide and is initially processed as with any digital image
with a dark frame subtraction. The image is then cropped into a 765 by 20 pixel
swath, which allows it to be imported into SPECTRA software. The image is then
expanded and calibrated with known emission lines from gas discharge tubes.
Alternatively, the calibration can be carried out directly on the spectral
features, provided that at least two lines can be identified. The expanded
spectrum can be further processed to highlight subtle line features.
(Click on image for enlarged illustration.)
|
Figure 2 shows the general procedure
utilized to obtain and process a spectrum. The initial spectrum consists of a swath
of light only a few pixels wide. Following dark subtraction, the image is cropped
to a 765x20 pixel area, which allows it to now be imported to SPECTRA for further
analysis, which includes wavelength calibration and expansion into the more
familiar "line spectrum". In addition, a calibrated text file can be saved and
imported into any one of a variety of graphic spreadsheets such as Excel, where
the flux calibration can be carried out. Once the spectrum is expanded into a line
profile, various routines can be applied which enhance the lines and make them
easier to identify (Figure 2). I have found that a gentle
sharpening routine and/or an unsharp mask produce a reasonably enhancement of
features with no artifact introduction. Absorption and emission line identifications
were carried out using tables from the Handbook of Chemistry and Physics [3].
Flux calibration, when required, was done according to published methods [4]
and is indicated in Figure 3. The method consists of using published absolute
fluxes per unit wavelength for standard spectrometric stars (Figure 3A) and
normalizing of spectra of the same standard stars obtained with my optical
arrangement (Figure 3B-D) against these values. This general procedure is outlined
in Figure 3.
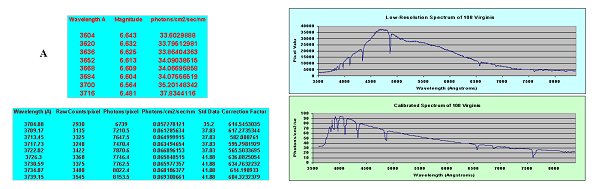
Figure 3.
Flux calibration of spectra. A. Digital flux calibration data for
standard stars is downloaded from appropriate database and is given as
magnitudes per wavelength interval. This is converted to photons/cm2/sec/nm
using the formula F = 5.5x106(1/ )10-0.4(mag). B. The spectra
of the same star is obtained with your particular optical system and converted
to photons/cm2/sec/nm. Division of the calibrated data by your results produces
a correction factor, which is then applied at the appropriate wavelength intervals. C. S
C. Spectrum of 108 Virginis before flux calibration and (D) after flux calibration.
(Click on image for enlarged illustration.)
|
Once the calibration has been carried out and a set of normalizing
values obtained, these values can be used indefinitely as long as your set up
remains unchanged. If simple identification of absorption or emission lines is all
that is required, flux calibration can be eliminated. However, if more robust
analysis of the data is to be carried out, flux calibration is essential.
For example, in order to determine temperatures and electron densities in emission
nebulae, flux calibration is required since a careful determination of line
intensity ratios are needed for these type calculations. This type of analysis
will be demonstrated later in this paper.
Results and Discussion
The low-resolution mode is useful for stellar classification and obtaining spectra
of planetary nebula. In the high-resolution mode, many absorption lines are visible
of atoms, ions and simple molecules.
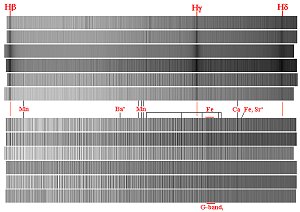
|
Figure 4.
Classification of stars based on their Spectra. Spectra were obtained in the
high-resolution mode from H to H for stars from early B to M class. Note the
increase followed by decrease in the hydrogen absorption lines as one proceeds
from B to M type stars along with the general increase in the number of metal
lines as the temperature decreases toward M class stars. Several different
metal lines are identified along with the G band representing the diatomic
molecule CH.
(Click on image for enlarged illustration.)
|
Figure 4 shows the higher resolution spectra
of stars from class B to M and luminosity class III and spans the region from
H to about 4100 A. Several of the more prominent lines are labeled. As one can
see, many lines are present, especially as one proceeds to cooler stars. Another
way this can be examined is shown in Figure 5, where the wavelength-calibrated
results were imported into Microsoft Excel for further analysis.
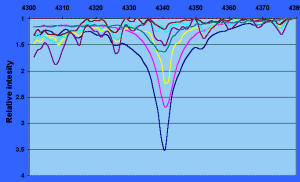
|
Figure 5.
Graphical representation of the spectral sequence centered around the
H-gamma absorption line. Note how the intensity of the H-gamma line, which has been
normalized to 1 for all spectra at 4380 Angstroms, increases to a maximum
at type A stars and gradually falls off with cooler stellar types. Several
lines for iron (Fe) and chromium (Cr) are also identified.
(Click on image for enlarged illustration.)
|
The region
centered around H (4300-4380 Å) has been normalized and expanded to show how
the H line profiles change with spectral type and how neutral Iron (Fe) and
Chromium (Cr) lines are affected by stellar type. The line profile of relatively
intense lines such as those for Hydrogen contains information regarding the
physics of the stellar atmosphere. For example, buried in the line profile are
such information as pressure, density and temperature, some of which, with the
appropriate mathematics, can be extracted. In addition, rotation of the star is
also contained within the profile, which also can be extracted [5].
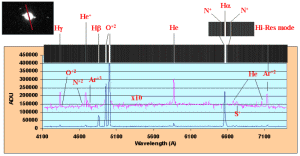
|
Figure 6.
Spectrum of planetary nebula NGC 7009 (Saturn Nebula). In the upper left
corner the positioning of the slit is indicated. The low-resolution spectra
is shown as both a graph and an emission line profile. The high resolution
spectrum is shown in the upper right centered around the H line showing the
presence of N+ lines straddling the H line. Various other ionic and atomic
species are identified. Many of these lines are forbidden such as the intense
5007 Angstrom line of O+2.
(Click on image for enlarged illustration.)
|
Figure 6 shows the emission line spectrum of NGC7009, The Saturn Nebula. The upper
left corner shows an image of the nebula with the slit superimposed over the nebula.
The main body of the line spectrum was obtained in the low-resolution mode so that
a majority of the emission features could be observed in a single picture. A higher
resolution spectrum is shown in the upper right centered at the H line. In
high-resolution mode, three components are seen to make up this strong emission, H
and two lines of N+, which flank H . A graphical presentation of the line spectra
is also shown. The usual types of species are apparent in the spectrum of a planetary
nebula. The Hydrogen and Helium lines along with the prominent forbidden O+2
lines, Ar+2, Ar+3, N+2 and S+ round out the variety of ionic species present.
For extended objects such as nebulae, one obtains a spectrum across the entire
length of the slit. During the analysis of the results, the software allows you
to select small sub-regions of the spectrum for analysis. As a result, one can
profile the composition across the entire slit, effectively obtaining many spectra
of the object at a single time, which is analyzed within the software. As discussed
below, this would also allow for profiling temperature and density of the nebula
across the slit.
In addition to identifying emission features, other physical features of a nebula
can be determined such as the temperature (T) and electron density (Ne).
Theoretically derived equations have been obtained which relate electron density
and temperature to line ratio intensities as seen in equations 1 and 2.
- (I4959 + I5007)/I4363 = [7.15/(1 + 0.00028Ne/T1/2)]1014300/T
- (I6548 + I6584)/I5755 = [8.50/(1 + 0.00290Ne/T1/2)]1010800/T
Equation 1 relates the line intensity ratios for transition occurring at 4959, 5007 and 4363
Angstroms for O+2 while equation 2 relates the lines at 6548, 6584 and 5755
Angstroms for N+. If your spectral analysis is isolated to a small region of
the nebula, you can assume that the electron density is the same in both
equations and combine them to yield equation 3, which is now independent of Ne.
- [(I6548 + I6584)/I5755]7.15x1014300/T – [(I4959 + I5007)/I4363]0.82x1010800/T =
0.9[(I6548 + I6584)/I5755] [(I4959 + I5007)/I4363]
One can determine the intensities of the lines from your spectrum, assuming you have
performed a flux calibration and use equation 3 to determine the temperature
of the nebula (note that equation 3 cannot be solved explicitly for temperature
but must be solved in an iterative fashion). As an example, for The Blue Snowball,
NGC 7662, [(I6548 + I6584)/I5755] = 45 and [(I4959 + I5007)/I4363] = 152. This
provides a temperature of 11,200°K (literature value 13,000°K [6]). Taking this
value for the temperature and using either equation 1 or 2 will give an electron
density of 30,000/cm3 (literature value 32,000/cm3 [6]). This example portrays
the gold mine of information contained within a spectrum. As was mentioned above,
for an extended object the spectrum across the entire slit is obtained and line
ratios as a function of distance from the central star, for example, can be
obtained in a single spectrum. The potential is present to create 2 dimensional
profiles of temperature, density and composition for nebular type
objects.
When the spectrometer is used in high-resolution mode, many absorption features
can be observed in the spectra, particularly in cooler stars. Simple image
processing techniques enhance these features making identification if features
much easier. Figure 7 shows the high-resolution spectra of 78 Virginis, an Ap
type star which exhibits enhanced quantities of Europium (Eu), Chromium (Cr)
and Strontium (Sr) in its outer atmosphere. A few of the many lines have been
identified and are labeled in the spectrum. Iron, Barium and Chromium are dominant
features. The identification of species responsible for the observed absorption
lines remains somewhat of an art. The Chemistry and Physics Handbook lists
over 21,000 lines between wavelengths of 3600 and 10000 Angstroms for all the
elements. This represents on average about six lines per Angstrom wavelength
interval. Since the instrument is only at best able to resolve 1-2 Angstroms in
the higher resolution mode, some criteria must be used to eliminate many of the
potential lines observed or as often the case, a line may represent a blend of
two or more lines.
The presence and intensity of a feature, due to an atom or ionic species, is the
result of many parameters such as natural abundance, probability of the absorption,
temperature, density and pressure. For our purposes, the first three are the most
important. Many potential features can be eliminated at the start simply because
the natural abundance of an element is so low. For example, the H absorption line
at 4861.33 Angstroms has a relative intensity of 80 compared to 3000 for
Protactinium at 4861.49 Angstroms. Yet one would not typically assign this
absorption line in a stellar spectrum to Protactinium simply because the natural
abundance of this element is 9 orders of magnitude lower than that of Hydrogen.
In addition to this abundance factor, other
absorption lines for Hydrogen are present where they should be and in the
appropriate intensity ratios whereas Protactinium lines are not. In order to be
certain of an assignment of a line, it is important to find several lines for a
particular species. Thus as you can see in figure 7, the lines for Europium,
Chromium and Strontium have many lines which are apparent for each species.
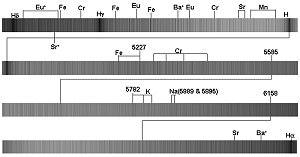
|
Figure 7.
The optical spectrum of 78 Virginis, an Ap star of the Europium-Chromium-Strontium
class. These type of stars exhibit enhanced abundance’s of these heavy metals and
the identification of absorption lines are labeled along with the identification
of more common metal lines such as Iron (Fe), Sodium (Na) and Potassium (K). The
numbers indicate the wavelengths of selected absorption lines in Angstroms.
Eu = Europium, Cr = Chromium, Sr = Strontium, Ba = Barium.
(Click on image for enlarged illustration.)
|
This makes you more confident that your assignment is real. In addition, for all the
spectra shown in this article, the spectra obtained were compared directly with
those obtained in the astronomical literature to be certain of the assignments.
My initial goals in using this instrument were to establish how far the instrument
could be pushed in giving spectra AND in the resulting identification of features.
Wolf-Rayet stars are massive stars (more than 25 solar masses), in an advanced stage
of evolution and ejecting at high speed a hot gas (103 km/s typically). They have
surface temperatures typically in the region of 100,000 K. The spectrum shows strong,
wide emission lines. Wolf-Rayet stars have lost their atmospheres and have exposed
their heavy cores. One finds the signature of ions of Helium, Carbon, Nitrogen and
Oxygen in the spectra.
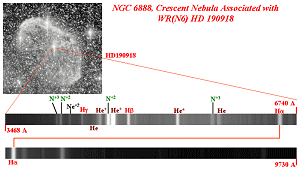
|
Figure 8.
Wolf-Rayet star HD 190918 (type WN) associated with NGC 6888, the Crescent
Nebula. Note the strong, broad lines of ionized Nitrogen and Helium
in emission, typical of these type stars.
(Click on image for enlarged illustration.)
|
There are 2 class Wolf-Rayet star: type WN shown in
Figure 8 where the ions of Helium and Nitrogen dominate and type WC, shown in
Figure 9, where ions of Carbon, Oxygen and Helium are the predominate species
observed.
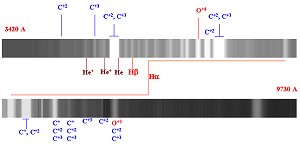
|
Figure 9.
Wolf-Rayet star HD 165763 (type WC). Notice the distinguishing feature of
these stars, the emission lines of multi-ionized states of Carbon and Oxygen.
The wavelength range spans from 3420Å at the upper left to 9730Å in the
lower right.
(Click on image for enlarged illustration.)
|
Figure 10 illustrates just how far this instrument is capable of being pushed to
give useful data. R Andromeda is an S3.5e-S8.8e (M7e) Mira type variable star
with a period of 409 days. Stars of this spectral class often exhibit absorption
lines due to the unstable element Technetium.
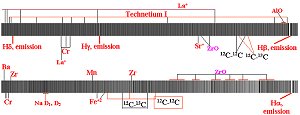
|
Figure 10.
The spectra of R And, an S3.5e-S8.8e (M7e) Mira type variable star with a
period of 409 days. The upper spectrum runs from 4100 Angstroms to 4900
Angstroms while the bottom spectrum runs from 5800 to 6600 Angstroms.
Note the presence of emission lines for
H , H , H and H lines along with
the presence of the unstable element technetium. In addition to enhanced
amounts of 13C as seen in diatomic carbon lines, zirconium oxide molecular
bands are also observed.
(Click on image for enlarged illustration.)
|
This element is not found naturally
in the solar system because it’s longest lived isotope, 97Te, has a half-life of
only 2.6 x 106 years and as a result all Technetium endogenous to the formation
of the solar system has long since decayed. Yet multiple lines of Technetium can
be detected in the atmosphere of this star and others of the S and C types [7].
Apparently, neutron capture is proceeding deep within stars of this type followed
by a dredging mechanism, which brings to the surface nuclear processed material.
Note also the presence of Zirconium and Zirconium Oxide molecules, which give rise
to extensive banded structure in the spectrum in the red region. The presence of
Zirconium Oxide bands is the defining feature of
S-type stars. In this particular S type star, emission lines are observed
super-imposed upon the more usual Hydrogen absorption lines.
The other very interesting aspect of these type stars is the fact that they
often contain abnormal amounts of 13C compared to 12C. The normal solar system
ratio of 12C to 13C is 80, but in many of these type stars this ratio can
approach 4. Normally, the detection of isotopes of atoms or ions cannot be
done easily because the lines are extremely close together and normal line
broadening effects cause them to overlap. However, this is not the case with
molecules where rotation and vibration transitions are much more sensitive to
the isotope composition. These types of transitions are normally outside the
optical range but electronic transitions can couple with vibration transitions
giving rise to absorption lines often observable in the optical region. In cooler
type S and C type stars diatomic Carbon forms and the clear separation of
absorption lines occurs between diatomic 12C-12C
and 12C-13C as is indicated
on the spectrum in Figure 10.
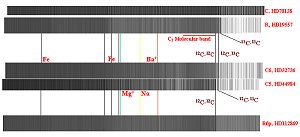
(Click on image for enlarged illustration.)
|
Figure 11.
Spectra of type C and R stars containing varying ratios of
12C to 13C. These stars are noted for their large quantities of
carbon as observed with diatomic carbon. The solar system value
for this ratio 80. The upper pair of spectra represents stars
with ratios in the area of 10. All three possible combinations
of C-C is observed, 12C-12C,
12C-13C and 13C-12C. The middle pair
represents stars with higher ratios such that the 13C-12C is no
longer observed and finally for stars approaching solar system ratios,
only 12C-12C is seen (lower spectrum. The blanketing effect of diatomic
carbon is shown along with the identification of several metal lines.
|
Figure 11 shows the spectra of a variety of type C and R stars containing varying
ratios of 12C to 13C. Some of these stars are noted for their large quantities of
carbon as observed with diatomic Carbon. In fact, these stars have C/O ratios >1
while "normal" stars are more in the range of 0.5-0.6. The upper pair of spectra
represents stars with ratios in the area of 10. All three possible combinations
of diatomic C-C are observed, 12C-12C,
12C-13C and
13C-13C. The middle pair of
spectra represents stars with higher ratios such that
the 13C-13C is no longer
clearly observed and finally for stars approaching solar system ratios,
only 12C-12C
is seen (lower spectrum). The blanketing effect of diatomic Carbon is shown along
with the identification of several metal lines. In addition, with careful wavelength
calibration one can measure the Doppler shift of absorption and emission lines to
determine velocities of approach or recession of objects along with rotation
velocities of stars and planets. I have been able to successfully observe and
measure the rotation of Saturn by aligning the slit along the rings of the planet.
In only a few second exposures one is able to obtain a spectrum of the entire disk
and ring system of Saturn. The eastern and western region of the image can be
isolated using SPECTRA software and each of the spectra calibrated against H line.
When the final two images are aligned they show the clear shift in the lines which
occurs due to Saturn s rotation from which can be calculated the rotation velocity.
In a similar manner, the velocity of approach of M31 has been determined by
acquiring the spectrum of the nucleus of the galaxy. In this case only the
narrow 18-µm slit was used and as a result a relatively long 60-minute exposure
was necessary. I have not tried using the wider slit but according to the
instrument specifications, again of 2 magnitudes is possible, with of course
a loss of resolution, which would occur with the wider slit, but one should be
able to determine the center of lines with the soft ware provided.
Future Directions
The future is certainly bright for amateur spectroscopy. As far as the SGS is
concerned, future versions will offer the option of having a higher dispersive
grating (1800 lines/mm). As of this writing, September 2001, this option will
be available within the next few months. This will improve in the identification
of lines by spreading the spectrum out by a factor of three compared to the
current high-resolution grating. In addition, user friendly and versatile software
for the amateur spectroscopist would be most welcome. While IRAF is the current
standard for this type work among professionals, it’s requirement of a Unix or
Linux operating system along with what I understand to be a difficult package
to learn at best will make this system little used by the budding spectroscopist.
To this end, perhaps the spectroscopy module currently being developed by Axiom
to be part of the MIRA software package will fill the void.
Conclusions
The Santa Barbara Instrument Group Spectrometer represents a quantum leap forward
for the amateur interested in the fertile area of spectroscopy. Even with a
relatively small telescope, this instrument coupled to sensitive CCD cameras
and utilizing the self-guiding feature of the ST-7/8 camera allows one to reach
unprecedented magnitudes and carry out spectral analysis only dreamed of by the
amateur a few years ago. Even after using the instrument for a year, I remain
astounded at the fact that an amateur with only relatively modest equipment from
his own backyard can detect Technetium, many dozens of other elements, simple
molecules and Carbon isotopes in stars or nebulae hundreds of light years away!
References
[1] SBIG web page www.sbig.com
[2] MaxIm, Cyanogen Productions Inc., www.cyanogen.com
[3] Handbook of Chemistry and Physics, 79th edition, 1998-1999, section 10-1 to 10-88.
[4] Getting the most from a CCD Spectrograph, S. Kannappan and D. Fabricant, S&T, 100(1), 125-132, 2000.
[5] Optical Astronomical Spectroscopy, C.R. Kitchin, Institute of Physics Publishing Ltd, 1995.
[6] Astrophysical Formula, K.R. Lang, Springer-Verlag, 2nd edition, 1980.
[7] The Classification of Stars, C. Jaschek and M. Jaschek, Cambridge University Press, 1987.
About the Author
Dale E. Mais - Mais Observatory
15219 Cool Valley Rd., Valley Center, CA 92082
Email: dmais@ligand.com
Website: members.cts.com/cafe/m/mais/
Dale has been involved in amateur astronomy most of his life. He is an Endocrinology
researcher working for a Bio-Tech company in the San Diego area. While his biology
and chemistry degrees serve him well in his professional life, it is his chemistry
backgound, which he is enjoying as applied to spectroscopy. He is fortunate to have
an observatory with a Celestron 14 as his primary instrument, CCD cameras and an
AstroPhysics 5.1 inch (which he waited patiently for 2 years to obtain). His
location of 12 miles from Mount Palomar means he benefits from the outstanding
seeing, and relatively dark skies which the Hale telescope benefits from. His
primary interest is spectroscopy and its application toward understanding the
composition and other physical parameters of astronomical objects. In particular,
he is doing a spectroscopic survey of C and S type stars, which often has abnormal
heavy metal and/or isotope composition compared to solar system values. In addition,
he is interested in quantitation of atomic/ionic species in stellar atmospheres.
- Ed. BDM
|